Reactive oxygen species in the regulation of synaptic plasticity and memory
- PMID: 20649473
- PMCID: PMC3078504
- DOI: 10.1089/ars.2010.3208
Reactive oxygen species in the regulation of synaptic plasticity and memory
Abstract
The brain is a metabolically active organ exhibiting high oxygen consumption and robust production of reactive oxygen species (ROS). The large amounts of ROS are kept in check by an elaborate network of antioxidants, which sometimes fail and lead to neuronal oxidative stress. Thus, ROS are typically categorized as neurotoxic molecules and typically exert their detrimental effects via oxidation of essential macromolecules such as enzymes and cytoskeletal proteins. Most importantly, excessive ROS are associated with decreased performance in cognitive function. However, at physiological concentrations, ROS are involved in functional changes necessary for synaptic plasticity and hence, for normal cognitive function. The fine line of role reversal of ROS from good molecules to bad molecules is far from being fully understood. This review focuses on identifying the multiple sources of ROS in the mammalian nervous system and on presenting evidence for the critical and essential role of ROS in synaptic plasticity and memory. The review also shows that the inability to restrain either age- or pathology-related increases in ROS levels leads to opposite, detrimental effects that are involved in impairments in synaptic plasticity and memory function.
Figures
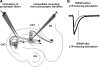
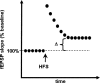
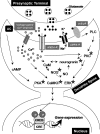
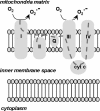
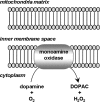
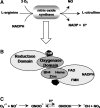
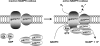
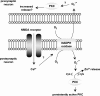
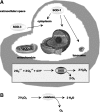
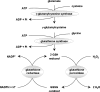
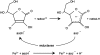
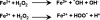
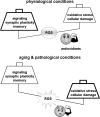
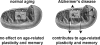
Similar articles
-
Neuronal and vascular oxidative stress in Alzheimer's disease.Curr Neuropharmacol. 2011 Dec;9(4):662-73. doi: 10.2174/157015911798376244. Curr Neuropharmacol. 2011. PMID: 22654724 Free PMC article.
-
Reactive oxygen species and synaptic plasticity in the aging hippocampus.Ageing Res Rev. 2004 Nov;3(4):431-43. doi: 10.1016/j.arr.2004.05.002. Ageing Res Rev. 2004. PMID: 15541710 Review.
-
Sources and targets of reactive oxygen species in synaptic plasticity and memory.Antioxid Redox Signal. 2007 Feb;9(2):233-44. doi: 10.1089/ars.2007.9.ft-8. Antioxid Redox Signal. 2007. PMID: 17115936 Free PMC article. Review.
-
A role for reactive oxygen/nitrogen species and iron on neuronal synaptic plasticity.Antioxid Redox Signal. 2007 Feb;9(2):245-55. doi: 10.1089/ars.2007.9.245. Antioxid Redox Signal. 2007. PMID: 17115937 Review.
-
Reactive Oxygen Species: Physiological and Physiopathological Effects on Synaptic Plasticity.J Exp Neurosci. 2016 Sep 4;10(Suppl 1):23-48. doi: 10.4137/JEN.S39887. eCollection 2016. J Exp Neurosci. 2016. PMID: 27625575 Free PMC article. Review.
Cited by
-
Mitochondrial Dysfunction as a Potential Mechanism Mediating Cardiac Comorbidities in Parkinson's Disease.Int J Mol Sci. 2024 Oct 12;25(20):10973. doi: 10.3390/ijms252010973. Int J Mol Sci. 2024. PMID: 39456761 Free PMC article. Review.
-
Pathophysiology and neuroprotection of global and focal perinatal brain injury: lessons from animal models.Pediatr Neurol. 2015 Jun;52(6):566-584. doi: 10.1016/j.pediatrneurol.2015.01.016. Epub 2015 Jan 31. Pediatr Neurol. 2015. PMID: 26002050 Free PMC article. Review.
-
Brain modulation by the gut microbiota: From disease to therapy.J Adv Res. 2023 Nov;53:153-173. doi: 10.1016/j.jare.2022.12.001. Epub 2022 Dec 7. J Adv Res. 2023. PMID: 36496175 Free PMC article. Review.
-
Weaving a Net of Neurobiological Mechanisms in Schizophrenia and Unraveling the Underlying Pathophysiology.Biol Psychiatry. 2016 Oct 15;80(8):589-98. doi: 10.1016/j.biopsych.2016.03.1047. Epub 2016 Mar 9. Biol Psychiatry. 2016. PMID: 27113498 Free PMC article. Review.
-
Improving behavioral deficits induced by perinatal ethanol and stress exposure in adolescent male rat progeny via maternal melatonin treatment.Psychopharmacology (Berl). 2024 Jan;241(1):153-169. doi: 10.1007/s00213-023-06470-z. Epub 2023 Oct 27. Psychopharmacology (Berl). 2024. PMID: 37889278
References
-
- Abdullaev JF. Caballero-Ortega H. Riveron-Negrete L. Pereda-Miranda R. Rivera-Luna R. Manuel HJ. Perez-Lopez I. Espinosa-Aguirre JJ. In vitro evaluation of the chemopreventive potential of saffron. Rev Invest Clin. 2002;54:430–436. - PubMed
-
- Abe K. Sugiura M. Shoyama Y. Saito H. Crocin antagonizes ethanol inhibition of NMDA receptor-mediated responses in rat hippocampal neurons. Brain Res. 1998;787:132–138. - PubMed
-
- Abraham WC. Williams JM. LTP maintenance and its protein synthesis-dependence. Neurobiol Learn Mem. 2008;89:260–268. - PubMed
-
- Adam K. Lack of effect on mental efficiency of extra vitamin C. Am J Clin Nutr. 1981;34:1712–1716. - PubMed
Publication types
MeSH terms
Substances
Grants and funding
LinkOut - more resources
Full Text Sources
Other Literature Sources
Medical
Research Materials