Abstract
A striking feature of the marine fossil record is the variable intensity of extinction during superficially similar climate transitions. Here we combine climate models and species trait simulations to explore the degree to which differing palaeogeographic boundary conditions and differing magnitudes of cooling and glaciation can explain the relative intensity of marine extinction during greenhouseâicehouse transitions in the Late Ordovician and the Cenozoic. Simulations modelled the response of virtual species to cooling climate using a spatially explicit cellular automaton algorithm. We find that palaeogeography alone may be a contributing factor, as identical changes in meridional sea surface temperature gradients caused greater extinction in Late Ordovician simulations than in Cenozoic simulations. Differences in extinction from palaeogeography are significant, but by themselves are insufficient to explain observed differences in extinction intensity. However, when simulations included inferred changes in continental flooding and interval-specific models of sea surface temperature, predicted differences in relative extinction intensity were more consistent with observations from the fossil record. Our results support the hypothesis that intense extinction in the Late Ordovician is partially attributable to exceptionally rapid and severe cooling compared to Cenozoic events.
This is a preview of subscription content, access via your institution
Access options
Access Nature and 54 other Nature Portfolio journals
Get Nature+, our best-value online-access subscription
$29.99 /Â 30Â days
cancel any time
Subscribe to this journal
Receive 12 print issues and online access
$259.00 per year
only $21.58 per issue
Buy this article
- Purchase on SpringerLink
- Instant access to full article PDF
Prices may be subject to local taxes which are calculated during checkout




Similar content being viewed by others
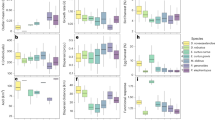
Data availability
Data from simulations are provided as Supplementary Data.
Code availability
Simulation code is provided as Supplementary Software.
References
Bambach, R. K. Phanerozoic biodiversity mass extinctions. Annu. Rev. Earth Planet. Sci. 34, 127â155 (2006).
Raup, D. M. & Sepkoski, J. J. Mass extinctions in the marine fossil record. Science 215, 1501â1503 (1982).
Bond, D. P. G. & Wignall, P. B. Large igneous provinces and mass extinctions: an update. Geol. Soc. Am. Spec. Pap. 505, 29â55 (2014).
Svensen, H. et al. Siberian gas venting and the end-Permian environmental crisis. Earth Planet. Sci. Lett. 277, 490â500 (2009).
Joachimski, M. M. & Buggisch, W. Conodont apatite δ18O signatures indicate climatic cooling as a trigger of the Late Devonian mass extinction. Geology 30, 711â714 (2002).
Brenchley, P. J. et al. High-resolution stable isotope stratigraphy of Upper Ordovician sequences: constraints on the timing of bioevents and environmental changes associated with mass extinction and glaciation. Geol. Soc. Am. Bull. 115, 89â104 (2003).
Melchin, M. J., Mitchell, C. E., Holmden, C. & Å torch, P. Environmental changes in the Late OrdovicianâEarly Silurian: review and new insights from black shales and nitrogen isotopes. Geol. Soc. Am. Bull. 125, 1635â1670 (2013).
Sheehan, P. M. The Late Ordovician mass extinction. Annu. Rev. Earth Planet. Sci. 29, 331â364 (2001).
Edie, S. M., Huang, S., Collins, K. S., Roy, K. & Jablonski, D. Loss of biodiversity dimensions through shifting climates and ancient mass extinctions. Integr. Comp. Biol. 58, 1179â1190 (2018).
Hansen, T. A. Extinction of Late Eocene to Oligocene molluscs: relationship to shelf area, temperature changes and impact events. Palaios 2, 69â75 (1987).
Ivany, L. C., Patterson, W. P. & Lohmann, K. C. Cooler winters as a possible cause of mass extinctions at the Eocene/Oligocene boundary. Nature 407, 887â890 (2000).
Powell, M. G. Timing and selectivity of the Late Mississippian mass extinction of brachiopod genera from the Central Appalachian Basin. Palaios 23, 525â534 (2008).
Stanley, S. M. Anatomy of a regional mass extinction: PlioâPleistocene decimation of the Western Atlantic bivalve fauna. Palaios 1, 17â36 (1986).
Bartlett, R. et al. Abrupt global-ocean anoxia during the Late OrdovicianâEarly Silurian detected using uranium isotopes of marine carbonates. Proc. Natl Acad. Sci. USA 115, 5896â5901 (2018).
Ghienne, J.-F. et al. A Cenozoic-style scenario for the End-Ordovician glaciation. Nat. Commun. 5, 4485 (2014).
Hammarlund, E. U. et al. A sulfidic driver for the End-Ordovician mass extinction. Earth Planet. Sci. Lett. 331â332, 128â139 (2012).
Harper, D. A. T., Hammarlund, E. U. & Rasmussen, C. M. Ã. End Ordovician extinctions: a coincidence of causes. Gondwana Res. 25, 1294â1307 (2014).
Vandenbroucke, T. R. A. et al. Metal-induced malformations in Early Palaeozoic plankton are harbingers of mass extinction. Nat. Commun. 6, 7966 (2015).
Zhou, L. et al. Changes in marine productivity and redox conditions during the Late Ordovician Hirnantian glaciation. Palaeogeogr. Palaeoclimatol. Palaeoecol. 420, 223â234 (2015).
Zou, C. et al. Ocean euxinia and climate change âdouble whammyâ drove the Late Ordovician mass extinction. Geology 46, 535â538 (2018).
Melott, A. L. et al. Did a gamma-ray burst initiate the Late Ordovician mass extinction? Int. J. Astrobiol. 3, 55â61 (2004).
Gong, Q. et al. Mercury spikes suggest volcanic driver of the OrdovicianâSilurian mass extinction. Sci. Rep. 7, 5304 (2017).
Jones, D. S., Martini, A. M., Fike, D. A. & Kaiho, K. A volcanic trigger for the Late Ordovician mass extinction? Mercury data from South China and Laurentia. Geology 45, 631â634 (2017).
Saupe, E. et al. Macroevolutionary consequences of profound climate change on niche evolution in marine molluscs over the past three million years. Proc. R. Soc. Lond. B 281, 20141995 (2014).
Peterson, A. T. Ecological niche conservatism: a timeâstructured review of evidence. J. Biogeogr. 38, 817â827 (2011).
Stigall, A. L. When and how do species achieve niche stability over long time scales? Ecography 37, 1123â1132 (2014).
Poloczanska, E. S. et al. Global imprint of climate change on marine life. Nat. Clim. Change 3, 919â925 (2013).
Sunday, J. M., Bates, A. E. & Dulvy, N. K. Thermal tolerance and the global redistribution of animals. Nat. Clim. Change 2, 686â690 (2012).
Clarke, A. & Crame, J. A. The Southern Ocean benthic fauna and climate change: a historical perspective. Philos. Trans. R. Soc. B 338, 299â309 (1992).
Barnes, D. K., Griffiths, H. J. & Kaiser, S. Geographic range shift responses to climate change by Antarctic benthos: where we should look. Mar. Ecol. Prog. Ser. 393, 13â26 (2009).
Valentine, J. W. Paleoecologic Molluscan Geography of the Californian Pleistocene 134 (Univ. California Press, 1961).
Brett, C. E., Hendy, A. J., Bartholomew, A. J., Bonelli, J. R. Jr & McLaughlin, P. I. Response of shallow marine biotas to sea-level fluctuations: a review of faunal replacement and the process of habitat tracking. Palaios 22, 228â244 (2007).
Valentine, J. W. Evolutionary Paleoecology of the Marine Biosphere (Prentice Hall, 1973).
Cocks, L. R. M. & Torsvik, T. H. Earth geography from 500 to 400 million years ago: a faunal and palaeomagnetic review. J. Geol. Soc. Lond. 159, 631â644 (2002).
Stanley, S. M. Thermal barriers and the fate of perched faunas. Geology 38, 31â34 (2010).
Lear, C. H., Bailey, T. R., Pearson, P. N., Coxall, H. K. & Rosenthal, Y. Cooling and ice growth across the EoceneâOligocene transition. Geology 36, 251â254 (2008).
Pusz, A. E., Thunell, R. C. & Miller, K. G. Deep water temperature, carbonate ion and ice volume changes across the EoceneâOligocene climate transition. Paleoceanogr. Paleoclimatol. 26, PA2205 (2011).
Miller, K. G. et al. Climate threshold at the EoceneâOligocene transition: Antarctic ice sheet influence on ocean circulation. Geol. Soc. Am. Spec. Pap. 452, 169â178 (2009).
Coxall, H. K., Wilson, P. A., Pälike, H., Lear, C. H. & Backman, J. Rapid stepwise onset of Antarctic glaciation and deeper calcite compensation in the Pacific Ocean. Nature 433, 53â57 (2005).
Finnegan, S. et al. The magnitude and duration of Late OrdovicianâEarly Silurian glaciation. Science 331, 903â906 (2011).
Trotter, J. A., Williams, I. S., Barnes, C. R., Lécuyer, C. & Nicoll, R. S. Did cooling oceans trigger Ordovician biodiversification? Evidence from conodont thermometry. Science 321, 550â554 (2008).
Liu, Z. H. et al. Global cooling during the EoceneâOligocene climate transition. Science 323, 1187â1190 (2009).
Herbert, T. D., Peterson, L. C., Lawrence, K. T. & Liu, Z. Tropical ocean temperatures over the past 3.5 million years. Science 328, 1530â1534 (2010).
Katz, M. E. et al. Stepwise transition from the Eocene greenhouse to the Oligocene icehouse. Nat. Geosci. 1, 329â334 (2008).
Rasmussen, C. M. Ã. & Harper, D. A. T. Did the amalgamation of continents drive the End Ordovician mass extinctions? Palaeogeogr. Palaeoclimatol. Palaeoecol. 311, 48â62 (2011).
Sunday, J. M., Bates, A. E. & Dulvy, N. K. Global analysis of thermal tolerance and latitude in ectotherms. Proc. R. Soc. Lond. B 278, 1823â1830 (2010).
Finnegan, S., Rasmussen, C. M. Ã. & Harper, D. A. T. Biogeographic and bathymetric determinants of brachiopod extinction and survival during the Late Ordovician mass extinction. Proc. R. Soc. B 283, 20160007 (2016).
Finnegan, S., Heim, N. A., Peters, S. E. & Fischer, W. W. Climate change and the selective signature of the Late Ordovician mass extinction. Proc. Natl Acad. Sci. USA 109, 6829â6834 (2012).
Finnegan, S., Rasmussen, C. M. Ã. & Harper, D. A. Identifying the most surprising victims of mass extinction events: an example using Late Ordovician brachiopods. Biol. Lett. 13, 20170400 (2017).
Deutsch, C., Ferrel, A., Seibel, B., Pörtner, H.-O. & Huey, R. B. Climate change tightens a metabolic constraint on marine habitats. Science 348, 1132â1135 (2015).
Penn, J. L., Deutsch, C., Payne, J. L. & Sperling, E. A. Temperature-dependent hypoxia explains biogeography and severity of end-Permian marine mass extinction. Science 362, eaat1327 (2018).
Torsvik, T. H. & Cocks, L. R. M. Earth History and Palaeogeography (Cambridge Univ. Press, 2016).
Reddin, C. J., Kocsis, Ã. T. & Kiessling, W. Climate change and the latitudinal selectivity of ancient marine extinctions. Paleobiology 45, 70â84 (2019).
OâDea, A. et al. Formation of the Isthmus of Panama. Science 2, e1600883 (2016).
Qiao, H., Saupe, E. E., Soberón, J., Peterson, A. T. & Myers, C. E. Impacts of niche breadth and dispersal ability on macroevolutionary patterns. Am. Nat. 188, 149â162 (2016).
Rangel, T. F. L., DinizâFilho, J. A. F. & Colwell, R. K. Species richness and evolutionary niche dynamics: a spatial pattern-oriented simulation experiment. Am. Nat. 170, 602â616 (2007).
Saupe, E. E. et al. Non-random latitudinal gradients in range size and niche breadth predicted by spatial patterns of climate. Glob. Ecol. Biogeogr. 28, 928â942 (2019).
Blakey, R. C. Colorado Plateau Geosystems, 1â1 (Deep Time Maps, 2016); https://deeptimemaps.com/
Scotese, C. Digital Paleogeographic Map Archive, CD-ROM (PALEOMAP Project, Scotese, 2001).
Harris, J. et al. in Petroleum Systems AnalysisâCase Studies: AAPG Memoir Vol. 114 (eds AbuAli, M. A., Moretti, I. & NordgÃ¥rd BolÃ¥s, H. M.) 37â60 (American Association of Petroleum Geologists, 2017).
Acknowledgements
We thank C. Myers, A. Townsend Peterson and J. Soberón for help with developing the initial simulation framework, from which simulations were launched. H.Q. was supported by the Natural Science Foundation of China (31772432). E.E.S. was supported by an EAR NSF Postdoctoral Fellowship and Leverhulme Grant #DGR01020. A.P., J.-B.L. and Y.D. thank the CEA/ CCRT for providing access to HPC resources of TGCC under allocation 2014-012212 made by GENCI. This is a contribution to IGCP Project-653, âThe Onset of the Great Ordovician Biodiversification Eventâ. A.F. and D.J.L. acknowledge funding from NERC through NE/K014757/1, NE/I005722/1, NE/I005714/1 and (P.J.V. also) NE/P013805/1. D.J.L. and P.J.V. acknowledge funding through ERC grant âThe Greenhouse Earth Systemâ (T-GRES, project reference 340923). A.F., A.T.K.-A., D.J.L. and P.J.V. are thankful for the use of the computational facilities of the Advanced Computing Research Centre, University of Bristol (http://www.bris.ac.uk/acrc) (Bluecrystal). S.F. acknowledges funding from the David and Lucile Packard Foundation. This is PBDB publication no. 353. We thank all contributors to the PBDB, with special thanks to the top 10 for our data download: A. Hendy, W. Kiessling, P. Wagner, S. Holland, M. Uhen, B. Kröger, J. Alroy, A. Miller, M. Clapham and J. Sessa.
Author information
Authors and Affiliations
Contributions
E.E.S. and S.F. designed the study, collected the data and wrote the manuscript. A.F., A.P., A.T.K.-A., D.J.L., J.-B.L., P.V. and Y.D. contributed climate modelling data. E.E.S. and H.Q. performed analyses. All authors read and commented on the manuscript.
Corresponding author
Ethics declarations
Competing interests
The authors declare no competing interests.
Additional information
Peer review information Primary Handling Editor: James Super.
Publisherâs note Springer Nature remains neutral with regard to jurisdictional claims in published maps and institutional affiliations.
Supplementary information
Supplementary Information
Supplementary methods, Tables 1â5 and Figs. 1â18.
Supplementary Software 1
Description of the simulation framework and code, and how to download and implement it on your own system.
Supplementary Data 1
Species-level output on extinction status from the simulations.
Rights and permissions
About this article
Cite this article
Saupe, E.E., Qiao, H., Donnadieu, Y. et al. Extinction intensity during Ordovician and Cenozoic glaciations explained by cooling and palaeogeography. Nat. Geosci. 13, 65â70 (2020). https://doi.org/10.1038/s41561-019-0504-6
Received:
Accepted:
Published:
Issue Date:
DOI: https://doi.org/10.1038/s41561-019-0504-6