Using Paramecium as a Model for Ciliopathies
- PMID: 34680887
- PMCID: PMC8535419
- DOI: 10.3390/genes12101493
Using Paramecium as a Model for Ciliopathies
Abstract
Paramecium has served as a model organism for the studies of many aspects of genetics and cell biology: non-Mendelian inheritance, genome duplication, genome rearrangements, and exocytosis, to name a few. However, the large number and patterning of cilia that cover its surface have inspired extraordinary ultrastructural work. Its swimming patterns inspired exquisite electrophysiological studies that led to a description of the bioelectric control of ciliary motion. A genetic dissection of swimming behavior moved the field toward the genes and gene products underlying ciliary function. With the advent of molecular technologies, it became clear that there was not only great conservation of ciliary structure but also of the genes coding for ciliary structure and function. It is this conservation and the legacy of past research that allow us to use Paramecium as a model for cilia and ciliary diseases called ciliopathies. However, there would be no compelling reason to study Paramecium as this model if there were no new insights into cilia and ciliopathies to be gained. In this review, we present studies that we believe will do this. For example, while the literature continues to state that immotile cilia are sensory and motile cilia are not, we will provide evidence that Paramecium cilia are clearly sensory. Other examples show that while a Paramecium protein is highly conserved it takes a different interacting partner or conducts a different ion than expected. Perhaps these exceptions will provoke new ideas about mammalian systems.
Keywords: Paramecium; cilia; ciliate; ciliopathy.
Conflict of interest statement
The authors declare no conflict of interest.
Figures
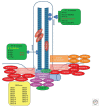
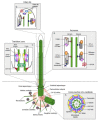
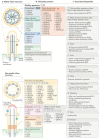
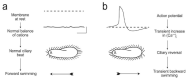
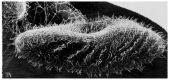
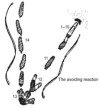
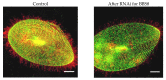
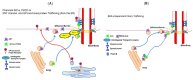
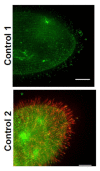
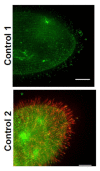
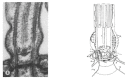
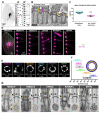
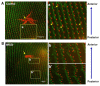
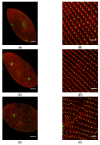
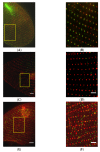
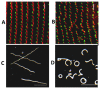
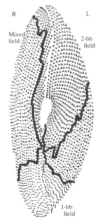
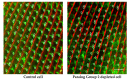
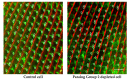
Similar articles
-
Paramecium, a Model to Study Ciliary Beating and Ciliogenesis: Insights From Cutting-Edge Approaches.Front Cell Dev Biol. 2022 Mar 14;10:847908. doi: 10.3389/fcell.2022.847908. eCollection 2022. Front Cell Dev Biol. 2022. PMID: 35359441 Free PMC article. Review.
-
Paramecium Biology.Results Probl Cell Differ. 2019;68:291-318. doi: 10.1007/978-3-030-23459-1_13. Results Probl Cell Differ. 2019. PMID: 31598862 Review.
-
[Paramecium, a model organism to study ciliogenesis and ciliopathies].Med Sci (Paris). 2021 Jun-Jul;37(6-7):632-638. doi: 10.1051/medsci/2021087. Epub 2021 Jun 28. Med Sci (Paris). 2021. PMID: 34180823 French.
-
Paramecium swimming and ciliary beating patterns: a study on four RNA interference mutations.Integr Biol (Camb). 2015 Jan;7(1):90-100. doi: 10.1039/c4ib00181h. Integr Biol (Camb). 2015. PMID: 25383612
-
Ciliary heterogeneity within a single cell: the Paramecium model.Methods Cell Biol. 2015;127:457-85. doi: 10.1016/bs.mcb.2014.12.007. Epub 2015 Feb 14. Methods Cell Biol. 2015. PMID: 25837404
Cited by
-
Structures and functions of cilia during vertebrate embryo development.Mol Reprod Dev. 2022 Dec;89(12):579-596. doi: 10.1002/mrd.23650. Epub 2022 Nov 11. Mol Reprod Dev. 2022. PMID: 36367893 Free PMC article. Review.
-
PCD Genes-From Patients to Model Organisms and Back to Humans.Int J Mol Sci. 2022 Feb 3;23(3):1749. doi: 10.3390/ijms23031749. Int J Mol Sci. 2022. PMID: 35163666 Free PMC article. Review.
-
Method for Stress Assessment of Endosymbiotic Algae in Paramecium bursaria as a Model System for Endosymbiosis.Microorganisms. 2022 Jun 18;10(6):1248. doi: 10.3390/microorganisms10061248. Microorganisms. 2022. PMID: 35744766 Free PMC article.
-
The Tubulin Superfamily in Apicomplexan Parasites.Microorganisms. 2023 Mar 9;11(3):706. doi: 10.3390/microorganisms11030706. Microorganisms. 2023. PMID: 36985278 Free PMC article.
-
A Review for the Special Issue on Paramecium as a Modern Model Organism.Microorganisms. 2023 Apr 3;11(4):937. doi: 10.3390/microorganisms11040937. Microorganisms. 2023. PMID: 37110360 Free PMC article. Review.
References
-
- Leeuwenhoek A. Observation, communicated to the publisher by Mr. Antony van Leewenhoek, in a Dutch letter of the 9 Octob. 1676. Philos. Trans. R. Soc. Lond. 1677;12:821–831. doi: 10.1098/4stl.1677.0003. - DOI
-
- Hill J. An History of Animals. Thomas Osborn Grays-Inn; London, UK: 1727.
Publication types
MeSH terms
LinkOut - more resources
Full Text Sources
Miscellaneous