EB1-microtubule interactions in Xenopus egg extracts: role of EB1 in microtubule stabilization and mechanisms of targeting to microtubules
- PMID: 12388761
- PMCID: PMC129970
- DOI: 10.1091/mbc.02-04-0210
EB1-microtubule interactions in Xenopus egg extracts: role of EB1 in microtubule stabilization and mechanisms of targeting to microtubules
Abstract
EB1 targets to polymerizing microtubule ends, where it is favorably positioned to regulate microtubule polymerization and confer molecular recognition of the microtubule end. In this study, we focus on two aspects of the EB1-microtubule interaction: regulation of microtubule dynamics by EB1 and the mechanism of EB1 association with microtubules. Immunodepletion of EB1 from cytostatic factor-arrested M-phase Xenopus egg extracts dramatically reduced microtubule length; this was complemented by readdition of EB1. By time-lapse microscopy, EB1 increased the frequency of microtubule rescues and decreased catastrophes, resulting in increased polymerization and decreased depolymerization and pausing. Imaging of EB1 fluorescence revealed a novel structure: filamentous extensions on microtubule plus ends that appeared during microtubule pauses; loss of these extensions correlated with the abrupt onset of polymerization. Fluorescent EB1 localized to comets at the polymerizing plus ends of microtubules in cytostatic factor extracts and uniformly along the lengths of microtubules in interphase extracts. The temporal decay of EB1 fluorescence from polymerizing microtubule plus ends predicted a dissociation half-life of seconds. Fluorescence recovery after photobleaching also revealed dissociation and rebinding of EB1 to the microtubule wall with a similar half-life. EB1 targeting to microtubules is thus described by a combination of higher affinity binding to polymerizing ends and lower affinity binding along the wall, with continuous dissociation. The latter is likely to be attenuated in interphase. The highly conserved effect of EB1 on microtubule dynamics suggests it belongs to a core set of regulatory factors conserved in higher organisms, and the complex pattern of EB1 targeting to microtubules could be exploited by the cell for coordinating microtubule behaviors.
Figures
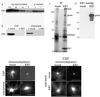
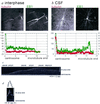
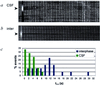
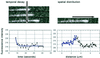
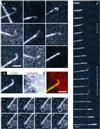
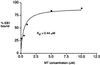
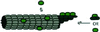
Similar articles
-
EB1 targets to kinetochores with attached, polymerizing microtubules.Mol Biol Cell. 2002 Dec;13(12):4308-16. doi: 10.1091/mbc.e02-04-0236. Mol Biol Cell. 2002. PMID: 12475954 Free PMC article.
-
EB1 regulates microtubule dynamics and tubulin sheet closure in vitro.Nat Cell Biol. 2008 Apr;10(4):415-21. doi: 10.1038/ncb1703. Epub 2008 Mar 23. Nat Cell Biol. 2008. PMID: 18364701
-
Microtubule plus-end tracking by CLIP-170 requires EB1.Proc Natl Acad Sci U S A. 2009 Jan 13;106(2):492-7. doi: 10.1073/pnas.0807614106. Epub 2009 Jan 6. Proc Natl Acad Sci U S A. 2009. PMID: 19126680 Free PMC article.
-
Structural basis of EB1 effects on microtubule dynamics.Biochem Soc Trans. 2009 Oct;37(Pt 5):997-1001. doi: 10.1042/BST0370997. Biochem Soc Trans. 2009. PMID: 19754439 Review.
-
Regulation of end-binding protein EB1 in the control of microtubule dynamics.Cell Mol Life Sci. 2017 Jul;74(13):2381-2393. doi: 10.1007/s00018-017-2476-2. Epub 2017 Feb 15. Cell Mol Life Sci. 2017. PMID: 28204846 Free PMC article. Review.
Cited by
-
Phosphorylation of EB2 by Aurora B and CDK1 ensures mitotic progression and genome stability.Nat Commun. 2016 Mar 31;7:11117. doi: 10.1038/ncomms11117. Nat Commun. 2016. PMID: 27030108 Free PMC article.
-
Aurora B spatially regulates EB3 phosphorylation to coordinate daughter cell adhesion with cytokinesis.J Cell Biol. 2013 May 27;201(5):709-24. doi: 10.1083/jcb.201301131. J Cell Biol. 2013. PMID: 23712260 Free PMC article.
-
Microtubule plus-end dynamics in Xenopus egg extract spindles.Mol Biol Cell. 2004 Apr;15(4):1776-84. doi: 10.1091/mbc.e03-11-0824. Epub 2004 Feb 6. Mol Biol Cell. 2004. PMID: 14767058 Free PMC article.
-
XMAP215-EB1 interaction is required for proper spindle assembly and chromosome segregation in Xenopus egg extract.Mol Biol Cell. 2009 Jun;20(11):2684-96. doi: 10.1091/mbc.e08-10-1051. Epub 2009 Apr 15. Mol Biol Cell. 2009. PMID: 19369422 Free PMC article.
-
Spindle fusion requires dynein-mediated sliding of oppositely oriented microtubules.Curr Biol. 2009 Feb 24;19(4):287-96. doi: 10.1016/j.cub.2009.01.055. Curr Biol. 2009. PMID: 19230671 Free PMC article.
References
-
- Akhmanova A, et al. Clasps are CLIP-115 and -170 associating proteins involved in the regional regulation of microtubule dynamics in motile fibroblasts. Cell. 2001;104:923–935. - PubMed
Publication types
MeSH terms
Substances
Grants and funding
LinkOut - more resources
Full Text Sources
Research Materials