Mechanism of spontaneous firing in dorsomedial suprachiasmatic nucleus neurons
- PMID: 15371499
- PMCID: PMC6729788
- DOI: 10.1523/JNEUROSCI.2146-04.2004
Mechanism of spontaneous firing in dorsomedial suprachiasmatic nucleus neurons
Abstract
We studied acutely dissociated neurons from the dorsomedial (shell) region of the rat suprachiasmatic nucleus (SCN) with the aim of determining the ionic conductances that underlie spontaneous firing. Most isolated neurons were spontaneously active, firing rhythmically at an average frequency of 8 +/- 4 Hz. After application of TTX, oscillatory activity generally continued, but more slowly and at more depolarized voltages; these oscillations were usually blocked by 2 microm nimodipine. To quantify the ionic currents underlying normal spontaneous activity, we voltage clamped cells using a segment of the spontaneous activity of each cell as voltage command and then used ionic substitution and selective blockers to isolate individual currents. TTX-sensitive sodium current flowed throughout the interspike interval, averaging -3 pA at -60 mV and -11 pA at -55 mV. Calcium current during the interspike interval was, on average, fourfold smaller. Except immediately before spikes, calcium current was outweighed by calcium-activated potassium current, and in current clamp, nimodipine usually depolarized cells and slowed firing only slightly (average, approximately 8%). Thus, calcium current plays only a minor role in pacemaking of dissociated SCN neurons, although it can drive oscillatory activity with TTX present. During normal pacemaking, the early phase of spontaneous depolarization (-85 to -60 mV) is attributable mainly to background conductance; cells have relatively depolarized resting potentials (with firing stopped by TTX and nimodipine) of -55 to -50 mV, although input resistance is high (9.5 +/- 4.1 GOmega). During the later phase of pacemaking (positive to -60 mV), TTX-sensitive sodium current is dominant.
Figures
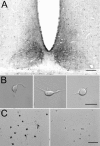
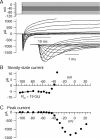
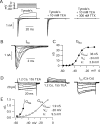
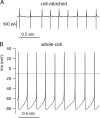
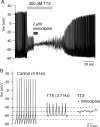
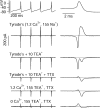
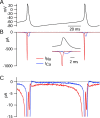
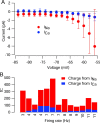
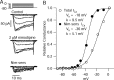
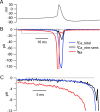
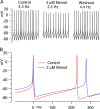
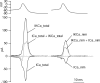
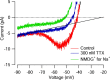
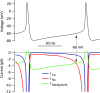
Similar articles
-
Sodium and calcium currents in acutely dissociated neurons from rat suprachiasmatic nucleus.J Neurophysiol. 1993 Oct;70(4):1692-703. doi: 10.1152/jn.1993.70.4.1692. J Neurophysiol. 1993. PMID: 7904302
-
Ionic currents underlying spontaneous action potentials in isolated cerebellar Purkinje neurons.J Neurosci. 1999 Mar 1;19(5):1663-74. doi: 10.1523/JNEUROSCI.19-05-01663.1999. J Neurosci. 1999. PMID: 10024353 Free PMC article.
-
Ionic currents and spontaneous firing in neurons isolated from the cerebellar nuclei.J Neurosci. 2000 Dec 15;20(24):9004-16. doi: 10.1523/JNEUROSCI.20-24-09004.2000. J Neurosci. 2000. PMID: 11124976 Free PMC article.
-
Cav1.3 Channels as Key Regulators of Neuron-Like Firings and Catecholamine Release in Chromaffin Cells.Curr Mol Pharmacol. 2015;8(2):149-61. doi: 10.2174/1874467208666150507105443. Curr Mol Pharmacol. 2015. PMID: 25966692 Free PMC article. Review.
-
Na+ currents that fail to inactivate.Trends Neurosci. 1993 Nov;16(11):455-60. doi: 10.1016/0166-2236(93)90077-y. Trends Neurosci. 1993. PMID: 7507618 Review.
Cited by
-
Intellectual disability-associated UNC80 mutations reveal inter-subunit interaction and dendritic function of the NALCN channel complex.Nat Commun. 2020 Jul 3;11(1):3351. doi: 10.1038/s41467-020-17105-8. Nat Commun. 2020. PMID: 32620897 Free PMC article.
-
Ionic mechanisms underlying autonomous action potential generation in the somata and dendrites of GABAergic substantia nigra pars reticulata neurons in vitro.J Neurosci. 2005 Sep 7;25(36):8272-81. doi: 10.1523/JNEUROSCI.1475-05.2005. J Neurosci. 2005. PMID: 16148235 Free PMC article.
-
Molecular and functional differences in voltage-activated sodium currents between GABA projection neurons and dopamine neurons in the substantia nigra.J Neurophysiol. 2011 Dec;106(6):3019-34. doi: 10.1152/jn.00305.2011. Epub 2011 Aug 31. J Neurophysiol. 2011. PMID: 21880943 Free PMC article.
-
The NALCN channel complex is voltage sensitive and directly modulated by extracellular calcium.Sci Adv. 2020 Apr 24;6(17):eaaz3154. doi: 10.1126/sciadv.aaz3154. eCollection 2020 Apr. Sci Adv. 2020. PMID: 32494638 Free PMC article.
-
Acute suppressive and long-term phase modulation actions of orexin on the mammalian circadian clock.J Neurosci. 2014 Mar 5;34(10):3607-21. doi: 10.1523/JNEUROSCI.3388-13.2014. J Neurosci. 2014. PMID: 24599460 Free PMC article.
References
-
- Akasu T, Shoji S, Hasuo H (1993) Inward rectifier and low-threshold calcium currents contribute to the spontaneous firing mechanism in neurons of the rat suprachiasmatic nucleus. Pflügers Arch 425: 109-116. - PubMed
-
- Bogdanov KY, Vinogradova TM, Lakatta EG (2001) Sinoatrial nodal cell ryanodine receptor and Na(+)-Ca(2+) exchanger: molecular partners in pacemaker regulation. Circ Res 88: 1254-1258. - PubMed
Publication types
MeSH terms
Substances
Grants and funding
LinkOut - more resources
Full Text Sources