Nanobatteries in redox-based resistive switches require extension of memristor theory
- PMID: 23612312
- PMCID: PMC3644102
- DOI: 10.1038/ncomms2784
Nanobatteries in redox-based resistive switches require extension of memristor theory
Abstract
Redox-based nanoionic resistive memory cells are one of the most promising emerging nanodevices for future information technology with applications for memory, logic and neuromorphic computing. Recently, the serendipitous discovery of the link between redox-based nanoionic-resistive memory cells and memristors and memristive devices has further intensified the research in this field. Here we show on both a theoretical and an experimental level that nanoionic-type memristive elements are inherently controlled by non-equilibrium states resulting in a nanobattery. As a result, the memristor theory must be extended to fit the observed non-zero-crossing I-V characteristics. The initial electromotive force of the nanobattery depends on the chemistry and the transport properties of the materials system but can also be introduced during redox-based nanoionic-resistive memory cell operations. The emf has a strong impact on the dynamic behaviour of nanoscale memories, and thus, its control is one of the key factors for future device development and accurate modelling.
Figures
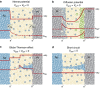




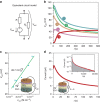

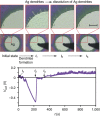
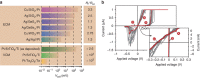
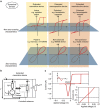
Similar articles
-
Recent Advances and Future Prospects for Memristive Materials, Devices, and Systems.ACS Nano. 2023 Jul 11;17(13):11994-12039. doi: 10.1021/acsnano.3c03505. Epub 2023 Jun 29. ACS Nano. 2023. PMID: 37382380 Review.
-
Stochastic memristive devices for computing and neuromorphic applications.Nanoscale. 2013 Jul 7;5(13):5872-8. doi: 10.1039/c3nr01176c. Epub 2013 May 22. Nanoscale. 2013. PMID: 23698627
-
Parylene-based memristive crossbar structures with multilevel resistive switching for neuromorphic computing.Nanotechnology. 2022 Mar 30;33(25). doi: 10.1088/1361-6528/ac5cfe. Nanotechnology. 2022. PMID: 35276689
-
Thousands of conductance levels in memristors integrated on CMOS.Nature. 2023 Mar;615(7954):823-829. doi: 10.1038/s41586-023-05759-5. Epub 2023 Mar 29. Nature. 2023. PMID: 36991190
-
Synapse-Mimetic Hardware-Implemented Resistive Random-Access Memory for Artificial Neural Network.Sensors (Basel). 2023 Mar 14;23(6):3118. doi: 10.3390/s23063118. Sensors (Basel). 2023. PMID: 36991829 Free PMC article. Review.
Cited by
-
Self-limited single nanowire systems combining all-in-one memristive and neuromorphic functionalities.Nat Commun. 2018 Dec 4;9(1):5151. doi: 10.1038/s41467-018-07330-7. Nat Commun. 2018. PMID: 30514894 Free PMC article.
-
Robust Memristor Networks for Neuromorphic Computation Applications.Materials (Basel). 2019 Oct 31;12(21):3573. doi: 10.3390/ma12213573. Materials (Basel). 2019. PMID: 31683537 Free PMC article.
-
Lithium ion trapping mechanism of SiO2 in LiCoO2 based memristors.Sci Rep. 2019 Mar 25;9(1):5081. doi: 10.1038/s41598-019-41508-3. Sci Rep. 2019. PMID: 30911041 Free PMC article.
-
Capacitive neural network with neuro-transistors.Nat Commun. 2018 Aug 10;9(1):3208. doi: 10.1038/s41467-018-05677-5. Nat Commun. 2018. PMID: 30097585 Free PMC article.
-
Demonstration of Synaptic Behaviors and Resistive Switching Characterizations by Proton Exchange Reactions in Silicon Oxide.Sci Rep. 2016 Feb 16;6:21268. doi: 10.1038/srep21268. Sci Rep. 2016. PMID: 26880381 Free PMC article.
References
-
- Aono M. & Hasegawa T.. The atomic switch. Proc. IEEE 98, 2228–2236 (2010).
-
- Valov I., Waser R., Jameson J. R. & Kozicki M. N. Electrochemical metallization memories-fundamentals, applications, prospects. Nanotechnology 22, 254003 (2011). - PubMed
-
- Waser R., Dittmann R., Staikov G. & Szot K. Redox-based resistive switching memories—nanoionic mechanisms, prospects, and challenges. Adv. Mater. 21, 2632–2663 (2009). - PubMed
-
- Borghetti J., Snider G. S., Kuekes P. J., Yang J. J., Stewart D. R. & Williams R. S. ‘Memristive’ switches enable ‘stateful’ logic operations via material implication. Nature 464, 873–876 (2010). - PubMed
-
- Fölling S., Türel Ö. & Likharev K. Single-electron latching switches as nanoscale synapses. Proc. IJCNN ’01 216–221 (2001).
Publication types
LinkOut - more resources
Full Text Sources
Other Literature Sources
Research Materials