Structure-guided unlocking of NaX reveals a non-selective tetrodotoxin-sensitive cation channel
- PMID: 35301303
- PMCID: PMC8931054
- DOI: 10.1038/s41467-022-28984-4
Structure-guided unlocking of NaX reveals a non-selective tetrodotoxin-sensitive cation channel
Abstract
Unlike classical voltage-gated sodium (NaV) channels, NaX has been characterized as a voltage-insensitive, tetrodotoxin-resistant, sodium (Na+)-activated channel involved in regulating Na+ homeostasis. However, NaX remains refractory to functional characterization in traditional heterologous systems. Here, to gain insight into its atypical physiology, we determine structures of the human NaX channel in complex with the auxiliary β3-subunit. NaX reveals structural alterations within the selectivity filter, voltage sensor-like domains, and pore module. We do not identify an extracellular Na+-sensor or any evidence for a Na+-based activation mechanism in NaX. Instead, the S6-gate remains closed, membrane lipids fill the central cavity, and the domain III-IV linker restricts S6-dilation. We use protein engineering to identify three pore-wetting mutations targeting the hydrophobic S6-gate that unlock a robust voltage-insensitive leak conductance. This constitutively active NaX-QTT channel construct is non-selective among monovalent cations, inhibited by extracellular calcium, and sensitive to classical NaV channel blockers, including tetrodotoxin. Our findings highlight a functional diversity across the NaV channel scaffold, reshape our understanding of NaX physiology, and provide a template to demystify recalcitrant ion channels.
© 2022. The Author(s).
Conflict of interest statement
C.L.N., M.K., T.C., C.T., J.T., C.A., C.C., and J.P. are or were employees of Genentech or Roche and own shares in the Genentech or Roche group. The remaining authors declare no competing interests.
Figures
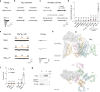
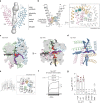
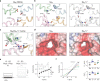
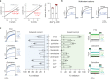
Similar articles
-
On the structural basis for size-selective permeation of organic cations through the voltage-gated sodium channel. Effect of alanine mutations at the DEKA locus on selectivity, inhibition by Ca2+ and H+, and molecular sieving.J Gen Physiol. 1997 Dec;110(6):693-715. doi: 10.1085/jgp.110.6.693. J Gen Physiol. 1997. PMID: 9382897 Free PMC article.
-
Use-dependent block of the voltage-gated Na(+) channel by tetrodotoxin and saxitoxin: effect of pore mutations that change ionic selectivity.J Gen Physiol. 2012 Oct;140(4):435-54. doi: 10.1085/jgp.201210853. J Gen Physiol. 2012. PMID: 23008436 Free PMC article.
-
Molecular pore structure of voltage-gated sodium and calcium channels.Braz J Med Biol Res. 1994 Dec;27(12):2781-802. Braz J Med Biol Res. 1994. PMID: 7550000 Review.
-
Ca2+ entry through NaV channels generates submillisecond axonal Ca2+ signaling.Elife. 2020 Jun 17;9:e54566. doi: 10.7554/eLife.54566. Elife. 2020. PMID: 32553116 Free PMC article.
-
The Nax (SCN7A) channel: an atypical regulator of tissue homeostasis and disease.Cell Mol Life Sci. 2021 Jul;78(14):5469-5488. doi: 10.1007/s00018-021-03854-2. Epub 2021 Jun 8. Cell Mol Life Sci. 2021. PMID: 34100980 Free PMC article. Review.
Cited by
-
Voltage-gated sodium channels: from roles and mechanisms in the metastatic cell behavior to clinical potential as therapeutic targets.Front Pharmacol. 2023 Jun 30;14:1206136. doi: 10.3389/fphar.2023.1206136. eCollection 2023. Front Pharmacol. 2023. PMID: 37456756 Free PMC article. Review.
-
Insights into the voltage-gated sodium channel, NaV1.8, and its role in visceral pain perception.Front Pharmacol. 2024 May 23;15:1398409. doi: 10.3389/fphar.2024.1398409. eCollection 2024. Front Pharmacol. 2024. PMID: 38855747 Free PMC article. Review.
-
The β3-subunit modulates the effect of venom peptides ProTx-II and OD1 on NaV 1.7 gating.J Cell Physiol. 2023 Jun;238(6):1354-1367. doi: 10.1002/jcp.31018. Epub 2023 Apr 12. J Cell Physiol. 2023. PMID: 37042220 Free PMC article.
-
NaX Channel Is a Physiological [Na+] Detector in Oxytocin- and Vasopressin-Releasing Magnocellular Neurosecretory Cells of the Rat Supraoptic Nucleus.J Neurosci. 2023 Dec 6;43(49):8306-8316. doi: 10.1523/JNEUROSCI.1203-23.2023. J Neurosci. 2023. PMID: 37783507 Free PMC article.
-
Structural biology and molecular pharmacology of voltage-gated ion channels.Nat Rev Mol Cell Biol. 2024 Nov;25(11):904-925. doi: 10.1038/s41580-024-00763-7. Epub 2024 Aug 5. Nat Rev Mol Cell Biol. 2024. PMID: 39103479 Review.
References
-
- Catterall WA, Goldin AL, Waxman SG. International Union of Pharmacology. XLVII. Nomenclature and structure-function relationships of voltage-gated sodium channels. Pharmacol. Rev. 2005;57:397–409. - PubMed
-
- Felipe A, Knittle TJ, Doyle KL, Tamkun MM. Primary structure and differential expression during development and pregnancy of a novel voltage-gated sodium channel in the mouse. J. Biol. Chem. 1994;269:30125–30131. - PubMed
-
- Akopian AN, Souslova V, Sivilotti L, Wood JN. Structure and distribution of a broadly expressed atypical sodium channel. FEBS Lett. 1997;400:183–187. - PubMed
-
- Hiyama TY, et al. Na(x) channel involved in CNS sodium-level sensing. Nat. Neurosci. 2002;5:511–512. - PubMed
Publication types
MeSH terms
Substances
LinkOut - more resources
Full Text Sources