Reversible Photocontrol of Dopaminergic Transmission in Wild-Type Animals
- PMID: 36077512
- PMCID: PMC9456102
- DOI: 10.3390/ijms231710114
Reversible Photocontrol of Dopaminergic Transmission in Wild-Type Animals
Abstract
Understanding the dopaminergic system is a priority in neurobiology and neuropharmacology. Dopamine receptors are involved in the modulation of fundamental physiological functions, and dysregulation of dopaminergic transmission is associated with major neurological disorders. However, the available tools to dissect the endogenous dopaminergic circuits have limited specificity, reversibility, resolution, or require genetic manipulation. Here, we introduce azodopa, a novel photoswitchable ligand that enables reversible spatiotemporal control of dopaminergic transmission. We demonstrate that azodopa activates D1-like receptors in vitro in a light-dependent manner. Moreover, it enables reversibly photocontrolling zebrafish motility on a timescale of seconds and allows separating the retinal component of dopaminergic neurotransmission. Azodopa increases the overall neural activity in the cortex of anesthetized mice and displays illumination-dependent activity in individual cells. Azodopa is the first photoswitchable dopamine agonist with demonstrated efficacy in wild-type animals and opens the way to remotely controlling dopaminergic neurotransmission for fundamental and therapeutic purposes.
Keywords: GPCR; azobenzene; behavior; brainwave; dopamine; in vivo electrophysiology; optogenetics; optopharmacology; photochromism; photopharmacology; photoswitch; zebrafish.
Conflict of interest statement
The authors declare no conflict of interest.
Figures

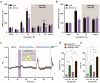
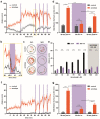
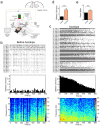
Similar articles
-
In vivo photocontrol of orexin receptors with a nanomolar light-regulated analogue of orexin-B.Cell Mol Life Sci. 2024 Jul 6;81(1):288. doi: 10.1007/s00018-024-05308-x. Cell Mol Life Sci. 2024. PMID: 38970689 Free PMC article.
-
Photocontrol of Endogenous Glycine Receptors In Vivo.Cell Chem Biol. 2020 Nov 19;27(11):1425-1433.e7. doi: 10.1016/j.chembiol.2020.08.005. Epub 2020 Aug 25. Cell Chem Biol. 2020. PMID: 32846115
-
Three-Photon Infrared Stimulation of Endogenous Neuroreceptors in Vivo.Angew Chem Int Ed Engl. 2023 Dec 18;62(51):e202311181. doi: 10.1002/anie.202311181. Epub 2023 Nov 20. Angew Chem Int Ed Engl. 2023. PMID: 37823736
-
Strategies and considerations of G-protein-coupled receptor photopharmacology.Adv Pharmacol. 2020;88:143-172. doi: 10.1016/bs.apha.2019.12.001. Epub 2020 Feb 10. Adv Pharmacol. 2020. PMID: 32416866 Review.
-
[Structural and functional organization of the cerebral dopaminergic system].Eksp Klin Farmakol. 2009 May-Jun;72(3):44-9. Eksp Klin Farmakol. 2009. PMID: 19642593 Review. Russian.
Cited by
-
In vivo photocontrol of orexin receptors with a nanomolar light-regulated analogue of orexin-B.Cell Mol Life Sci. 2024 Jul 6;81(1):288. doi: 10.1007/s00018-024-05308-x. Cell Mol Life Sci. 2024. PMID: 38970689 Free PMC article.
-
Unveiling the Neural Environment in Cancer: Exploring the Role of Neural Circuit Players and Potential Therapeutic Strategies.Cells. 2023 Aug 3;12(15):1996. doi: 10.3390/cells12151996. Cells. 2023. PMID: 37566075 Free PMC article. Review.
-
Light-Controlled Modulation and Analysis of Neuronal Functions.Int J Mol Sci. 2022 Oct 26;23(21):12921. doi: 10.3390/ijms232112921. Int J Mol Sci. 2022. PMID: 36361710 Free PMC article.
-
Light-Based Anti-Biofilm and Antibacterial Strategies.Pharmaceutics. 2023 Aug 9;15(8):2106. doi: 10.3390/pharmaceutics15082106. Pharmaceutics. 2023. PMID: 37631320 Free PMC article. Review.
References
-
- Firsov M.L., Astakhova L.A. The Role of Dopamine in Controlling Retinal Photoreceptor Function in Vertebrates. Neurosci. Behav. Phys. 2015;46:138–145. doi: 10.1007/s11055-015-0210-9. - DOI
MeSH terms
Substances
Grants and funding
- Human Brain Project WaveScalES, Specific Grant Agreement 2 No. 785907; NEUROPA project, Grant Agreement No. 863214/EU Horizon 2020 Framework Programme for Research and Innovation
- CERCA Programme/AGAUR/Generalitat de Catalunya
- Grant no. SAF2017-87629-R to V.C., SAF2016-80726-R to M.V.P., SAF2017-88076-R to M.M./Ministerio de Economía y Competitividad and European Regional Development Funds
- Grant no. 2017-SGR-1497 to V.C., 2017-SGR-210 to M.V.P., and 2017-SGR-00465 to J.H./Generalitat de Catalunya
- CECH project/European Union Regional Development Fund within the framework of the ERDF Operational Program of Catalonia 2014-2020
LinkOut - more resources
Full Text Sources
Molecular Biology Databases